The CLOUD Experiment
CLOUD Collaboration:
Aerodyne Research Inc., Billerica, Massachusetts 01821, USA
California Institute of Technology, Div. of Chemistry & Chemical Engineering, Pasadena, California 91125, USA
Carnegie Mellon University, Center for Atmospheric Particle Studies, Pittsburgh PA 15213-3890, USA
CERN, CH-1211 Geneva, Switzerland
Finnish Meteorological Institute, FI-00101 Helsinki, Finland
Goethe-University of Frankfurt, Institute for Atmospheric and Environmental Sciences, 60438 Frankfurt am Main, Germany
Helsinki Institute of Physics, University of Helsinki, FI-00014 Helsinki, Finland
Karlsruhe Institute of Technology, Institute for Meteorology and Climate Research, 76344 Eggenstein-Leopoldshafen, Germany
Lebedev Physical Institute, Solar and Cosmic Ray Research Laboratory, 119991 Moscow, Russia
Leibniz Institute for Tropospheric Research, 04318 Leipzig, Germany
Paul Scherrer Institute, Laboratory of Atmospheric Chemistry, CH-5232 Villigen, Switzerland
TOFWERK AG, CH-3600 Thun, Switzerland
University of Eastern Finland, Department of Applied Physics, FI-70211 Kuopio, Finland
University of Helsinki, Department of Physics, FI-00014 Helsinki, Finland
University of Innsbruck, Institute for Ion and Applied Physics, 6020 Innsbruck, Austria
University of Leeds, School of Earth and Environment, LS2-9JT Leeds, UK
University of Lisbon and University of Beira Interior, 1749-016 Lisbon, Portugal
University of Manchester, School of Earth, Atmospheric and Environmental Sciences, Manchester M13 9PL, UK
University of Stockholm, Department of Applied Environmental Science, 10691 Stockholm, Sweden
University of Vienna, Faculty of Physics, 1090 Vienna, Austria
1 INTRODUCTION
Aerosol-cloud interactions have a major influence on climate. Aerosol particles affect cloud droplet concentrations and, in turn, influence cloud reflectivity, precipitation, lifetime, dynamics and electrification. Changes of aerosol particles in the 20th century due to anthropogenic activities are thought to have offset a large fraction of the warming due to increased greenhouse gases [1]. However, aerosol-cloud processes are poorly understood and constitute the largest present uncertainty in radiative forcing of climate, severely limiting our ability to make accurate projections of future climate [2].
Up to half of all cloud droplets are estimated to form on cloud condensation nuclei (CCN) that were nucleated from trace vapours rather than being directly emitted into the atmosphere as particles [3]. The CLOUD experiment aims to resolve the fundamental physical and chemical processes involved in the formation of cloud-active aerosols, their growth to CCN sizes and their interaction with haze and clouds (Fig. 1). The experiment will measure, in particular, the influence of ions on these processes. Since ions in the free troposphere and the marine boundary layer result mainly from galactic cosmic rays (GCRs), their role in atmospheric nucleation is of considerable interest as a possible physical mechanism for solar-climate variability [4].
Fig. 1: Present and future CLOUD experiments on ion-aerosol-cloud processes in the laboratory from the nanometer molecular scale to the meter cloud scale.
2 CLOUD EXPERIMENT
The CLOUD chamber (Fig. 2) is a 3 m-diameter electropolished stainless steel cylinder (26.1 m3). The large size allows aerosol nucleation and growth processes to be studied at atmospheric concentrations, which are characterised by slow collision rates and growth rates. One metre diameter manholes are located at the top and bottom of the chamber to allow internal access. The chamber is equipped with numerous ports to allow installation of electrical/optical feedthroughs and sampling probes to extract air samples. Two transparent electrodes operating at voltages up to 30 kV are used to simulate an ion-free environment. The contents of the chamber can be irradiated by ultra violet (UV) light in the range 250-400 nm. The UV is introduced via 240 optical fibre vacuum feedthroughs installed on the top plate. These provide a uniform UV irradiation to generate photolytic (oxidation) reactions similar to those caused by sunlight, but without any parasitic heat load on the chamber. All materials and procedures used for the chamber are chosen to suppress contaminant vapours at the technological limit.
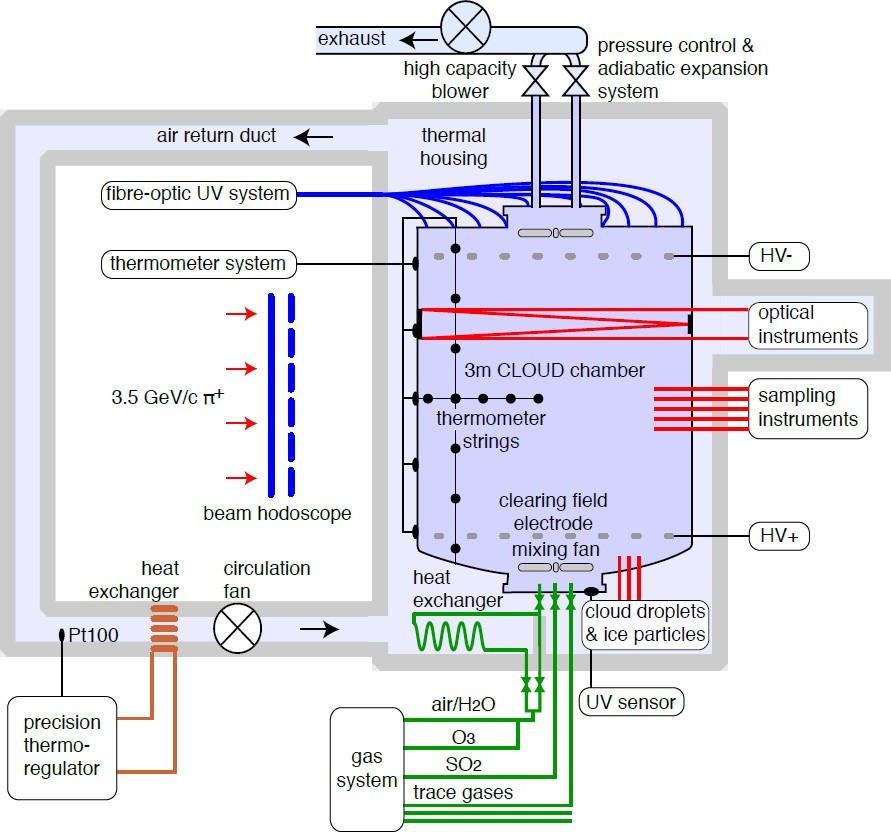
The chamber normally operates at +5 mbar relative to atmospheric pressure. However the chamber and gas system are designed to operate at up to +200 mbar relative pressure and to make controlled adiabatic expansions down to +5 mbar. In this way, starting from relative humidities near 100%, the chamber can be operated as a classical Wilson cloud chamber for studies of ion-aerosol interactions with cloud droplets and ice particles.
The chamber is surrounded by an insulated thermal housing. The temperature is controlled by precisely regulating the temperature of the air circulating in the space between the chamber and the thermal housing. Experimental runs can be performed at highly-stable temperatures (near 0.01C) between 40C and -70C. In addition, the temperature can be raised to 100C for bakeout. The chamber is exposed to a 3.5 GeV/c secondary π+ beam from the CERN PS, corresponding to the characteristic energies and ionisation densities of cosmic ray muons in the lower troposphere. The beam intensity can be adjusted to generate an ion-pair concentration spanning the atmospheric range from ground level to the stratosphere.
Ultra-pure synthetic air is obtained from the evaporation of cryogenic liquid N2 and liquid O2, mixed in the ratio 79:21, respectively. The air is humidified using ultra-pure water from a filtered recirculation system. A new ultra-pure water source is being developed to deliver synthetic water from burning electrolytically-produced H2 in pure O2 at 1400C. Ozone is added to the chamber by UV irradiation of a small inlet flow of dry air. Each trace gas delivered to the chamber has a dedicated line and isolation valve located at the chamber entrance, under the lower manhole cover. Magnetically coupled stainless steel fans on both manhole covers mix the fresh gases and the ions generated by the beam, and ensure uniformity inside the chamber. Volatile trace gases such as SO2 or NH3 are supplied from concentrated gas cylinders pressurised with N2 carrier gas. The trace gas mixtures are highly diluted using synthetic air before injection into the chamber. Less volatile trace gases such as α-pinene or pinanediol are supplied from temperature-controlled stainless steel evaporators using ultrapure N2 carrier gas. Chemically-produced trace vapours such as nitrous acid are supplied from custom-built stainless steel reactors. In order to compensate for sampling losses, there is a continuous flow of fresh gases into the chamber of up to 140 l/min, resulting in a dilution lifetime of about 3 h. A comprehensive array of state-of-the-art instruments continuously samples and analyses the contents of the chamber (Fig. 3).
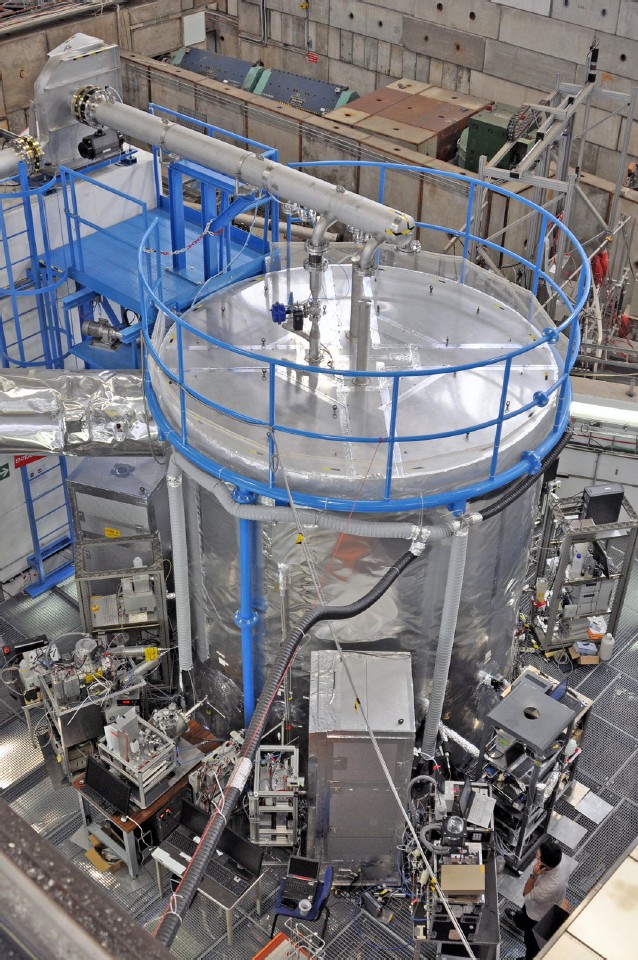
3 EXPERIMENTAL PROGRAMME
CLOUD has been in operation at CERN since November 2009. So far there have been two runs per year, each of around 2 months, during which CLOUD collects data continuously, 24 hours per day. Each afternoon during a run the findings from the previous day’s data are discussed in the CLOUD control room and the chamber conditions decided for the next 24 hours of data. These “3 o’clock meetings” are experimental science at its best.
The main focus during the seven CLOUD runs so far has been aerosol particle nucleation and growth, and the effect of ions on these processes (Table 1). Binary and ammonia ternary nucleation were studied during the first three runs (CLOUD1–3). These measurements were extended to free tropospheric conditions (temperatures down to 208 K) in CLOUD5. Nucleation and growth in the presence of oxidised organic species was studied in CLOUD4 (dimethylamine and pinanediol) and CLOUD7 (dimethylamine and α-pinene).
Campaign |
Month |
Year |
Aim |
CLOUD1 |
Nov-Dec |
2009 |
Commissioning |
CLOUD2 |
Jun-Jul |
2010 |
Binary H2SO4, NH3 ternary nucleation |
CLOUD3 |
Oct-Nov |
2010 |
Binary H2SO4, NH3 ternary nucleation |
CLOUD4 |
Jun-Jul |
2011 |
Dimethylamine & pinanediol ternary nucleation |
CLOUD5 |
Oct-Nov |
2011 |
Free tropospheric binary H2SO4, NH3 ternary nucleation |
CLOUD6 |
Jun-Jul |
2012 |
Initial cloud formation experiments |
CLOUD7 |
Oct-Dec |
2012 |
NH3, dimethylamine & α-pinene ternary nucleation & growth |
During CLOUD6 the first technical tests were made of a new system for controlled adiabatic pressure reductions (or, equivalently, volume expansions) inside the CLOUD chamber to create liquid and ice clouds (Fig. 4). Initial studies were carried out on cloud formation in the chamber at temperatures down το the homogeneous freezing point of water near 237 K (Fig. 5). Following this successful commissioning, a two-month physics run (CLOUD8) is planned for October–November 2013 to study ion-aerosol-cloud processes using cosmic rays.
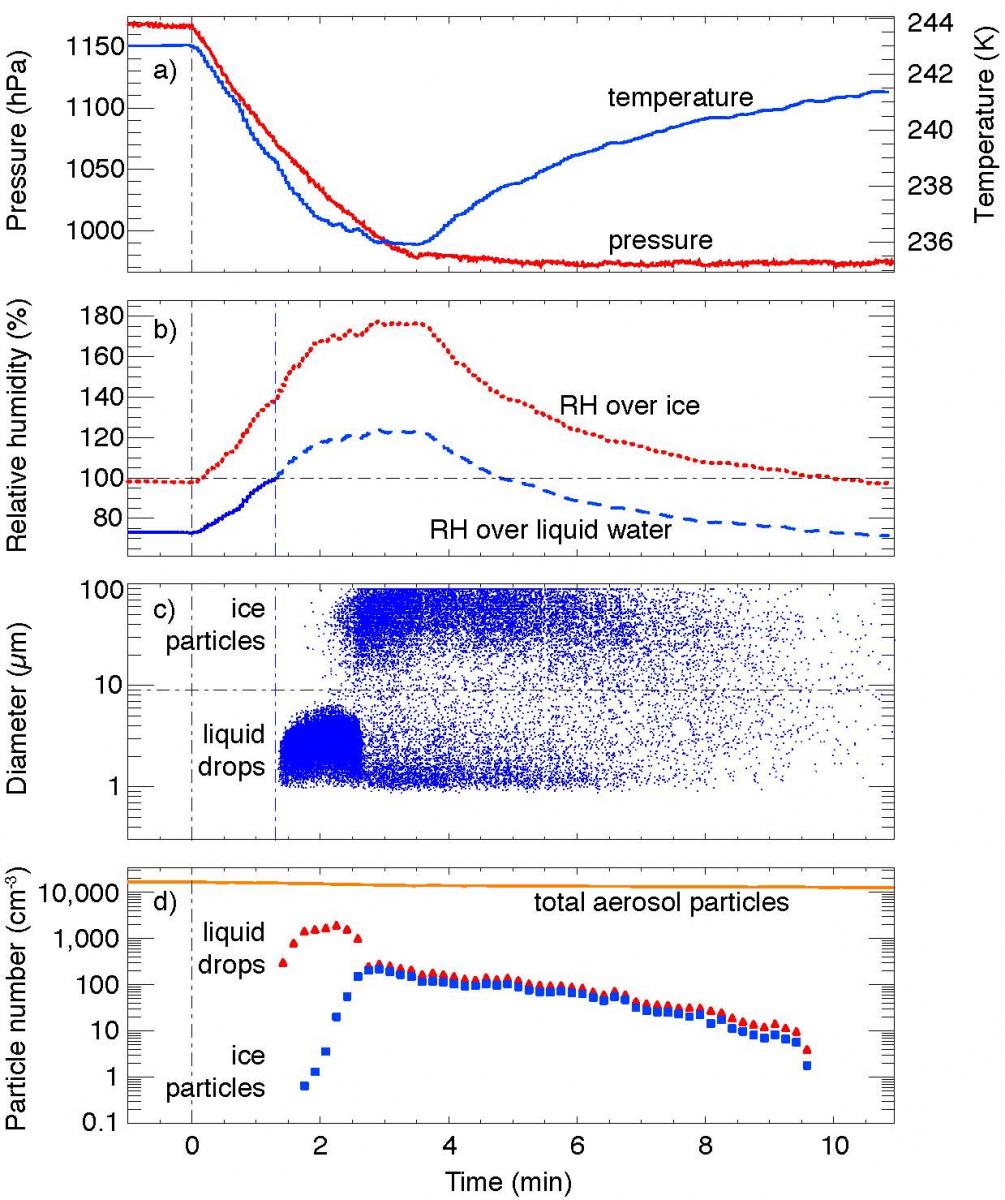
The CLOUD7 run involved the most complex series of experiments carried out so far. Around 25 sampling instruments were attached to the CLOUD chamber, including 9 state-of-art mass spectrometers to provide molecular analysis of vapours and nucleating clusters (Fig. 3). Several of these instruments were completely new and had only been in operation for a few weeks. The primary goal of CLOUD7 was to investigate aerosol particle nucleation and growth involving α-pinene, which is an important biogenic monoterpene emitted by forests and frequently found in the boundary layer at mixing ratios of a few 100 pptv or more. Monoterpenes are oxidised in the atmosphere, producing low volatility oxidised organic compounds that play an important role in atmospheric aerosols (contributing to the familiar blue haze seen when viewing distant mountains). In order to carry out the CLOUD7 experiments, several new ultra-clean gas sources were developed for CLOUD, including α-pinene (0–1200 pptv), nitrous acid (0–1000 pptv), and hydrogen (0.1%).
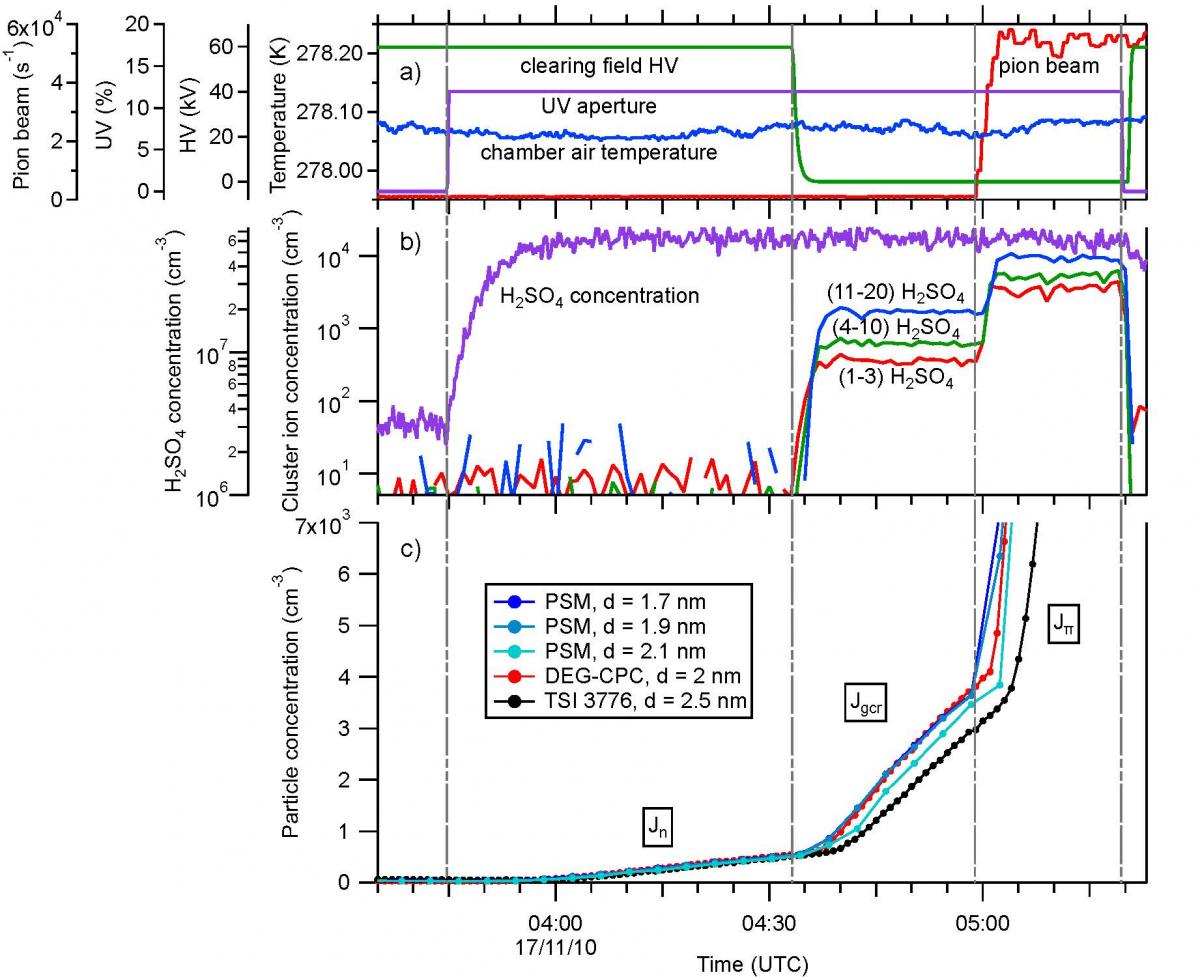
4 RESULTS
A typical measurement sequence from an early CLOUD run is shown in Fig. 6. The nucleation rates are measured under neutral (Jn), ground-level galactic cosmic ray (Jgcr), or charged pion beam (Jπ) conditions. Neutral nucleation rates are measured without any beam and with the electric clearing field on. For GCR and beam conditions, the electric field is set to zero, leading to ion pair concentrations around 400 cm-3 for Jgcr, representative of the boundary layer, and around 3000 cm-3 for Jπ, representative of the top of the troposphere.
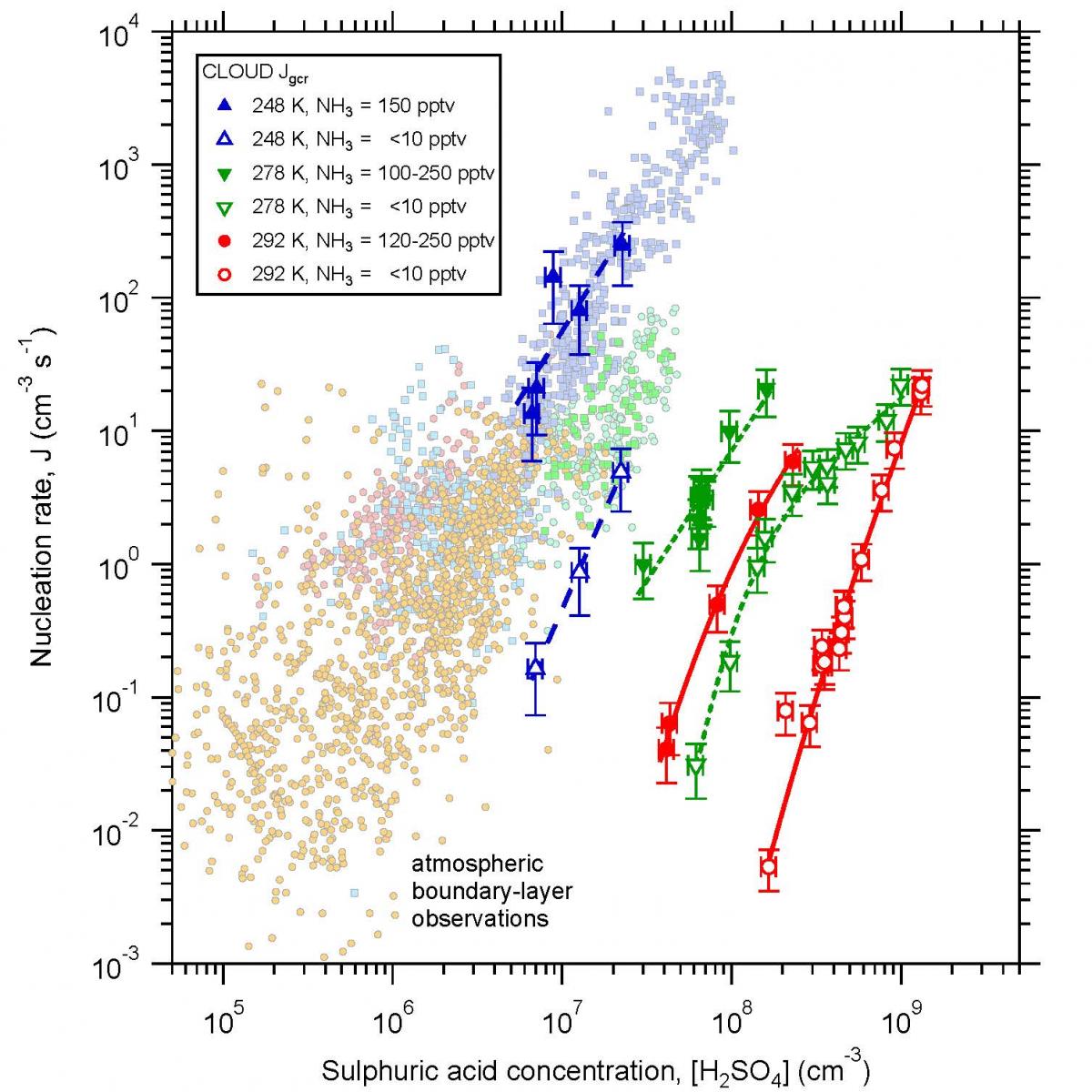
The first results from CLOUD showed that binary nucleation of H2SO4 - H2O particles does not take place in the boundary layer except under extremely cold conditions [5]. The presence of around 100 pptv NH3 was found to enhance the nucleation rates by about a factor 100; while higher amounts of NH3 resulted only in small further increases in rate. Ions corresponding to ground-level GCR fluxes produced a strong enhancement of the nucleation rates by up to a factor 2–10, depending on conditions.
However, even with ion enhancement, ammonia ternary nucleation still fails to explain atmospheric observations by a factor 10–100 or more (Fig. 7). The conclusion from the first CLOUD results is that organic vapours must be participating in boundary layer nucleation. This motivated the CLOUD4 and CLOUD7 runs, which studied dimethylamine (C2H7N), α-pinene (C10H16) and a closely-related species, pinanediol (C10H18O2). One of the important results from the first CLOUD measurements was the discovery of the base-stabilisation mechanism for ammonia ternary nucleation, involving the formation of strongly bound acid-base pairs which reduce evaporation from the cluster [5]. Dimethylamine was selected in these subsequent studies since it is expected to have stronger acid-base cluster binding energies than NH3 [6]. Alpha-pinene was selected as a principal species of biogenic monoterpene. The initial (CLOUD4) measurements of nucleation in the presence of oxidised organics were made with pinanediol in order to control the start of nucleation using UV (unlike α-pinene, the ozonolysis rate of pinanediol is very slow since there is no C=C double bond). An example of some ion-induced nucleation events (‘bananas’) from CLOUD7 is shown in Fig. 8.
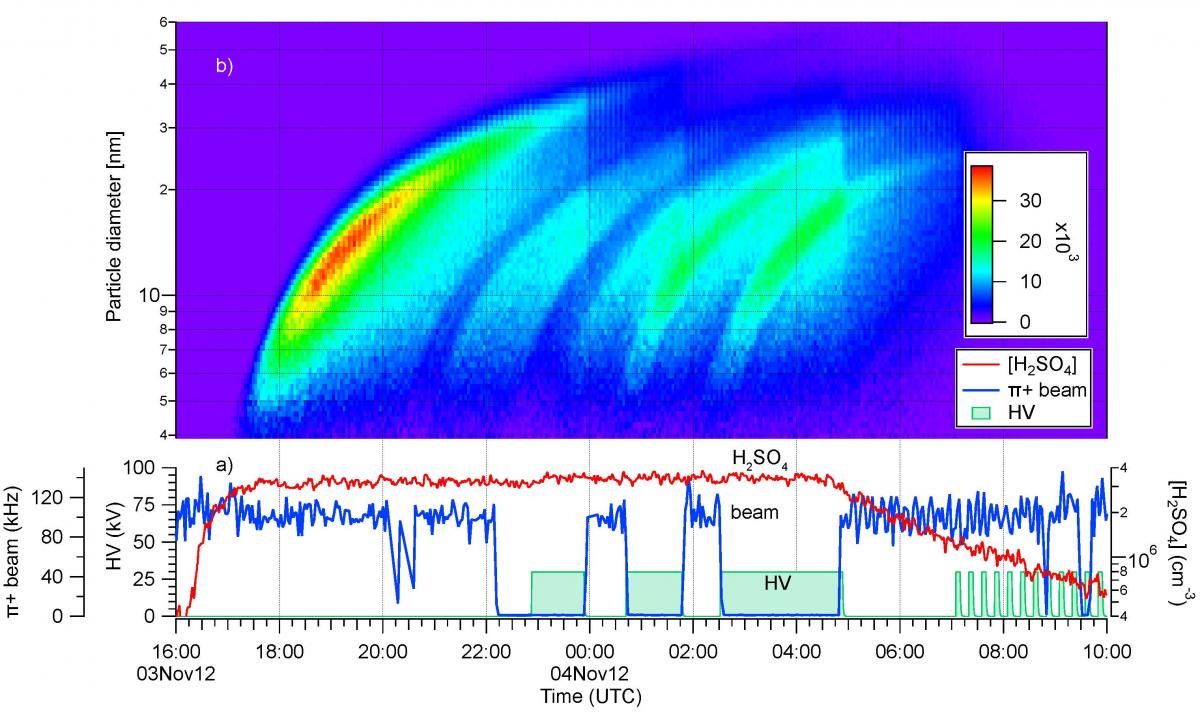
5 FUTURE PROSPECTS
Interpretation of observations made in the atmosphere of aerosol particle nucleation and growth is hindered by the presence of many sources of variability and ‘spectator’ background vapours. Laboratory experiments like CLOUD - which is recognised as the world’s most sophisticated laboratory experiment to study atmospheric nucleation [7] - are made under clean and precisely- controlled conditions, and can therefore identify the vapours responsible for the nucleation and growth processes, reveal the underlying molecular mechanisms and quantify the rates. However, the parameter space is so large that laboratory experiments must be guided by atmospheric observations and theoretical work in order to select the most promising vapours and conditions for detailed study. Laboratory experiments and field observations are therefore complementary and essential for each other [7].
The ultimate goals of CLOUD are twofold. Firstly, CLOUD aims to reach an improved understanding of the effect of atmospheric nucleation on global aerosol and clouds, and hence reduce the present uncertainties for aerosol radiative forcing of climate, and sharpen the projections of future climate change. Secondly, CLOUD aims to settle the question of whether or not cosmic rays exert a significant effect on aerosols, clouds and climate. To do this requires a close interaction between, on the one hand, the laboratory and field measurements and, on the other hand, global models that incorporate ions, aerosol and clouds at the microphysical level. In particular, the models need to identify at the process level the key sources of uncertainty in the radiative forcing of climate by aerosols in order to help focus the experimental measurements on reducing these uncertainties [8].
The question of whether, and to what extent, the climate is influenced by solar and cosmic ray variability remains vital for our understanding of the anthropogenic contribution to present climate change. Real progress on the cosmic ray-climate question will require a climatically-significant physical mechanism to be established, or else ruled out. The CERN CLOUD experiment aims to provide a definitive answer to this question over the next few years by studying the effects of ions on aerosol nucleation and growth and on cloud microphysical processes.
CLOUD at the 19th International Conference on Nucleation and Atmospheric Aerosols, ICNAA 2013
24-28 June 2013, Fort Collins, Colorado
http://chem.atmos.colostate.edu/icnaa/
This year's main conference on atmospheric aerosols is hosting a Special Session during the entire first day of the conference devoted to results from the CLOUD (Cosmics Leaving OUtdoor Droplets) experiment at CERN. The first two invited plenary talks of the conference will be presented by CLOUD scientists:
http://chem.atmos.colostate.edu/icnaa/ICNAA_2013_Program_sized.pdf